Inhibition of Nuclear Factor Kappa B (NF-B):
An Emerging Theme in Anti-Inflammatory Therapies
- Fulvio D'Acquisto, PhD,
- Michael J. May, PhD and
- Sankar Ghosh, PhD
- Section of Immunobiology and Department of Molecular Biophysics and Biochemistry Howard Hughes Medical Institute Yale University School of Medicine New Haven, CT 06510
- SG. E-mail sankar.ghosh{at}yale.edu; fax 203-737-1764.
Abstract
The application of anti-inflammatory therapies began thousands of years ago with the use of readily available natural resources. It is only recently, however, that the cellular and molecular mechanisms of inflammation have been appreciated sufficiently to design anti-inflammatory strategies with limited side effects. For example, salicylates and glucocorticoids, two widely used anti-inflammatory drug classes, are now known to inhibit the activation of NF-κB, a transcription factor that regulates the inducible expression of a wide range of proinflammatory mediators. New generations of NF-κB–targeting anti-inflammatory agents that are specific, efficacious, and cost-effective may therefore complement or replace current therapies. In this review, we describe various classes of NF-κB inhibitors and discuss important unresolved issues regarding their use.
In response to a variety of extracellular proinflammatory signals, nuclear factor (NF)-κB is translocated from the cell cytoplasm
into the nucleus. The cells shown above have been treated with TNF-α, in response to which NF-κB (stained red) becomes concentrated
in cell nuclei so as to activate the transcription of numerous genes that support an inflammatory response.
Introduction
On January 23, 1899, the dye factories of Friederich Bayer and Co. commercialized one of its most promising pain-relieving drugs (acetylsalicylic acid; ASA) under the name Aspirin (derived from “A” for acetyl, and “spirin” for spireic acid, a synonym of salicylic acid). However, the therapeutic uses and medical history of salicylates actually predate Bayer's product launch by many centuries (1). For example, in the time of the ancient Greeks, Hippocrates described the use of “lymph,” an extract of willow bark (now known to be a rich source of salicylic acid), for pain relief during labor. Remarkably, over 2000 years later, salicylates remain one of our most widely used anti-inflammatory therapies.
In the first century ad, Celsius described the major clinical symptoms of inflammation: dolor (pain),rubor (redness), tumor (swelling), and calor (heat). The molecular mechanisms responsible for the development of these symptoms are now understood to result from the enhanced expression of a subset of genes that normally maintain physiological homeostasis. For example, soluble mediators such as nitric oxide (NO), prostaglandins (PGs), tumor necrosis factor-α (TNF-α ), and interleukin-1 (IL-1) usually play a role in controlling important functions such as the regulation of blood pressure, platelet aggregation, and body temperature. Under pathologically inflammatory conditions, however, the production of these molecules promotes events ranging from increased leukocyte infiltration and vascular permeability to organ failure (2–5) . The selective inhibition of these and other inflammatory activities remains an important goal for the effective treatment of inflammation.
The induction of proinflammatory genes usually results from the increased activity of a subset of transcription factors that have thus become attractive targets in the development of novel anti-inflammatory drugs. Among the inducible transcription factors that control inflammatory gene expression, nuclear factor (NF)-κB plays a central and evolutionarily conserved role in coordinating the expression of various soluble proinflammatory mediators (e.g., cytokines and chemokines) and leukocyte adhesion molecules (6). In nonstimulated cells, NF-κB is sequestered within the cytosol by an inhibitory protein (i.e., IκB; inhibitor of NF-κB) that masks the nuclear localization signal present within the NF-κB protein sequence. Treatment of cells with proinflammatory cytokines such as TNF-α and IL-1, or with bacterial products such as lipopolysaccharide (LPS), leads to the activation of a specific IκB-kinase (IKK) complex that phosphorylates IκB and thereby tags it for ubiquitination and degradation by the proteasome (6,7). The degradation of IκB thus allows NF-κB to translocate into the nucleus where it can act as a transcription factor (Figure 1⇓).
In the absence of inflammatory activity, the transcription factor NF-κB is retained in the cytoplasm by a protein inhibitor (IkB). Proinflammatory stimuli activate a specific protein kinase, resulting in the degradation of IκB (pink) and translocation of NF-κB into the nucleus. (Details are elaborated in Figure 3.)
The IKK complex, as a critical activator of NF-κB function, has been the focus of intense research over the last several years. The complex is now known to consist of a core of three subunits, two of which, namely, IKKα and IKKβ contain functional kinase domains and are capable of phosphorylating IκB at specific N-terminal serine residues to initiate its ubiquitination. In contrast, the third core subunit of the IKK complex, called NEMO (NF-κB essential modulator; also known as IKKγ, or IKKAP), is a non-catalytic component that functions as a key regulator of IKK activity (7); the specific mechanism of NEMO regulatory activity remains poorly understood.
Following activation of the IKK complex and subsequent degradation of IκB, liberated NF-κB translocates to the nucleus and binds to specific elements (κB-sites) within the promoters of responsive genes to activate their transcription. A wide range of genes involved in inflammation contain functional κB-sites within their promoters and are induced by NF-κB (8). Thus, the findings that salicylates and glucocorticoids can inhibit NF-κB activity are among a growing body of data indicating that drugs specifically designed to target NF-κB activation might be clinically useful for the treatment of diseases that involve inflammation (Figure 2⇓) (9–13).
Diseases and conditions that may be alleviated by specific NF-κB–inhibiting drugs. Evidence from many distinct in vivo models demonstrates that the development of drugs selectively targeting NF-κB activity may yield pharmacologically relevant and therapeutically valuable treatments for a wide range of diseases and conditions in which inflammation plays a critical role. Some of the diseases that have been effectively treated by inhibitors of NF-κB in animal models are depicted.
CLASSES OF NF-kB–INHIBITING DRUGS
Aspirin and Salicylates
In 1971, John Vane demonstrated that acetylsalicylic acid (ASA) functions as an anti-inflammatory agent by inhibiting cyclooxygenase (COX), an enzyme activity essential to the production of PGs (14). Two isoforms of COX have since been identified, and whereas COX-1 is constitutively expressed in most tissues, COX-2 is generally induced by mitogens, cytokines, and bacterial products (15). ASA inhibits COX-1 activity by acetylating the enzyme; however, two general questions remain regarding the anti-inflammatory mechanism of salicylates. First, the anti-inflammatory effect of sodium salicylate cannot be attributed to covalent modification of COX-1, and second, the doses of ASA necessary to treat chronic inflammatory diseases are much higher than those required to inhibit PG synthesis. It therefore appears that a PG-independent pathway may be targeted in high-dose ASA experiments; indeed, we have found both ASA and sodium salicylate to inhibit the activation of NF-κB (10).
More recent studies demonstrate that the mechanism of inhibition by both ASA and sodium salycilate occurs by means of binding and blocking the ATP binding site of IKKβ (16). Intriguingly, expression of the COX-2–encoding gene, believed to be responsible for the massive production of PGs at inflammatory sites, is transcriptionally regulated by NF-κB (17, 18). Therefore, the decreased levels of PGs observed following treatment with high concentrations of ASA might be due to the reduced expression of COX-2 in addition to the inactivation of the COX-1 isoform. Both ASA and sodium salicylate inhibit NF-κB activation at doses comparable to the concentrations measured in the serum of patients treated with these agents for chronic inflammatory conditions.
The ability of ASA and sodium salicylate to inhibit NF-κB activity and κB-dependent gene expression has been tested experimentally in a wide range of cell types and conditions. For example, pretreatment of primary synovial fibroblasts or lung epithelial cells with ASA blocks IL-1– and TNF-α–induced NF-κB activation so that the subsequent expression of IL-6 and IL-8 is inhibited (19, 20). Similarly, treatment of human umbilical vein endothelial cells (HUVEC) with ASA inhibits TNF-α–induced NF-κB activity so as to block expression of leukocyte adhesion molecules, vascular cell adhesion molecule 1, and E-selectin (an endothelial-specific adhesion molecule). As a functional consequence of this inhibition, adhesion of U937 monocytes to TNF-α–stimulated HUVEC is markedly reduced (21). After treatment with ASA, dendritic cells, which are immunostimulatory bone marrow–derived cells, fail to undergo normal maturation, exhibit impaired LPS-induced expression of IL-12 and IL-10, and cannot mount normal cell-mediated contact hypersensitivity in vivo (22). Inhibition of NF-κB by ASA also prevents IL-12 production in human monocytes, and blocks development of the Th1 subset of T-cells (23). Thus, in addition to its anti-inflammatory functions vis-à-vis COX inhibition, ASA can exert potent immunomodulatory effects by blocking NF-κB activation and concomitant expression of critical immunoregulatory genes. The experimental systems in which salycilates act as inhibitors of NF-κB are summarized in Table 1⇓.
NF-kB Inhibitors that Demonstrate Anti-Inflammatory Activity in Experimental Models
Nonsteroidal Anti-Inflammatory Drugs
Following the discovery that salicylates can inhibit NF-κB activation, several laboratories set out to determine whether other nonsteroidal anti-inflammatory drugs (NSAIDs) act similarly. Surprisingly, several other drugs inhibit NF-κB activity at concentrations comparable to those used in therapy. Ibuprofen inhibits NF-κB activation in T cells (24) as well as COX-2 expression and PGE2 production in murine macrophages (Table 1⇑) (25). Furthermore, although the R stereoisomer of flurbiprofen has been considered “inactive” because it does not inhibit COX activity, it can nevertheless inhibit NF-κB activation both in vitro and in vivo (26). Among the other NSAIDs that have been investigated, acetaminophen inhibits the binding of NF-κB to DNA in LPS- and interferon-γ–treated macrophages and thus precludes expression of inducible nitric oxide synthase (27). Moreover, sulindac, an NSAID that is both structurally and pharmacologically related to indomethacin, decreases IKKβ kinase activity and consequently inhibits NF-κB activation (28). Lastly, tepoxalin, which inhibits both COX and 5-lipoxygenase, blocks NF-κB activation in Jurkat T-cells and HeLa cells. In contrast to these effects, naproxen, a COX inhibitor, and zileuton, an inhibitor of 5-lipoxygenase, do not affect NF-κB activity (29).
Glucocorticoids
Glucocorticoids (GCs) and other corticosteroids are the most widely used anti-inflammatory, immunosuppressive drugs. GCs function to downregulate the expression of genes involved in inflammation (30). In 1995, two separate groups of investigators demonstrated that GCs increase the expression of an IκB molecule, named IκBα, so that NF-κB is retained in the cytoplasm and thus rendered inactive (9, 11). Consistent with these findings, GCs fail to inhibit the binding of NF-κB to DNA in the presence of the protein synthesis inhibitor cycloheximide.
Subsequent studies, however, have challenged the role of GCs in upregulating the expression of IκBα, and alternative mechanisms have been proposed for the GC-mediated inhibition of NF-κB. In particular, mutational analysis of the glucocorticoid receptor, along with ligand binding studies, suggests that hormone-induced IκBα synthesis and inhibition of NF-κB activity are distinct biochemical processes. Thus, mutations that disrupt the dimeric nature of the glucocorticoid receptor and concomitantly impair the inducibility of IκBα expression can nevertheless function to repress NF-κB activity. Conversely, glucocorticoid analogs that enhance IκBα synthesis do not always repress NF-κB activity (31).
In addition the above effects of GCs, various alternative mechanisms that could account for their ability to inhibit NF-κB have been demonstrated experimentally. These include a direct physical interaction between NF-κB and the GC-occupied GC receptor in the nucleus (32–36); sequestration of p65-associated PKAc (i.e., the catalytic subunit of protein kinase A; p65 is also known as RelA) (37); inhibition of the phosphorylation of RNA polymerase II (38); and inhibition of NF-κB–associated histone acetyltransferase activity via recruitment of histone deacetlyase 2 (39). These mechanisms may thus act not to block NF-κB translocation to the nucleus, but rather to inhibit its ability to transactivate the expression of proinflammatory genes.
The notion that NF-κB inhibition underlies the anti-inflammatory capacity of GCs is also supported by animal studies. In a mouse model of acute inflammation (i.e., carrageenin-induced air pouch inflammation), treatment with the synthetic steroid dexamethasone reduces the number of cells that stain positively for activated NF-κB (40). In rats, dexamethasone not only inhibits TNF-α production and hepatic NF-κB activation associated with peritoneal sepsis (41), but also inhibits LPS-induced activation of NF-κB, restores myocardial contractility, and increases myocardial IκBα protein levels (Table 1⇑) (42). Finally, a recent study of normal human subjects demonstrates that intravenous injection of hydrocortisone increases IκBα levels and reduces the translocation of NF-κB into the nuclei of peripheral mononuclear cells (43), which supports the experimental model originally proposed for the molecular action of GCs (9, 11).
Antioxidants
The generation of reactive oxygen species (ROS) by phagocytic leukocytes (neutrophils, monocytes, macrophages, and eosinophils) is one of the most important hallmarks of the inflammatory process. By oxidizing essential cellular components of invading pathogens, reactive radicals and oxidants also represent the first line of defense against microorganisms (44). In addition to promoting general cytotoxicity, ROS may also act to upregulate proinflammatory gene expression by activating NF-κB, a process that is itself sensitive to the cellular redox state (45).
Diverse agents that cause oxidative stress can activate NF-κB (46), and numerous stimuli that activate NF-κB, including cytokines, phorbol esters, LPS, and CD3 engagement, increase the levels of intracellular ROS (47). This generation of ROS, however, is cell- and stimulus-specific. Thus, whereas stimulation with the proinflammatory cytokines IL-1 and TNF-α leads to substantial intracellular ROS in lymphoid and monocytic cell lines, no such increases have been observed in epithelial cell lines derived from ovaries, colon, breast, or cervix (47). Although evidence for the role of ROS in proinflammatory NF-κB activation remains circumstantial, more convincing studies demonstrate that a variety of antioxidant molecules, such as N-acetylcysteine, dithiocarbamates, vitamin E derivatives, and glutathione peroxidase, can inhibit NF-κB activation by inflammatory stimuli.
The potential therapeutic value of antioxidants as inhibitors of NF-κB has been demonstrated in a number of animal models of inflammatory disease (Table 1⇑). Dithiocarbamate not only inhibits NF-κB to alleviate IL-1– and LPS-mediated hypotension (i.e., an indicator of systemic inflammation), but also decreases the activation of NF-κB in tissues including the heart, lung, liver, and aorta (48, 49). Similarly, treatment of rats with N-acetylcysteine suppresses LPS-mediated activation of NF-κB activity in neutrophilic lung inflammation (50). The inhibition of NF-κB activation may thus be an important strategy for the treatment of sepsis-induced multiple organ injury (51–53). The potent antioxidant pyrrolidine dithiocarbamate markedly inhibits NF-κB activation in the spinal cord and attenuates the clinical symptoms of experimental allergic encephalomyelitis in rats (54).
Proteasome Inhibitors
A key step in the activation of NF-κB is the proteasome-dependent degradation of IκBα, which is initiated by phosphorylation of serine residues Ser32 and Ser36 by the IKK complex (55). Several inhibitors of proteasome activity, including lactacystin, the peptide aldehydes PSI and MG132, N-tosyl-lysine chloromethylketone (TLCK in Table 1⇑) and N-tosyl-phenylalanine chloromethylketone (TPCK in Table 1⇑) are all effective NF-κB inhibitors as well as potent anti-inflammatory drugs (56–58). In animal models of inflammation, specific protease inhibitors may block LPS-induced NF-κB activation as well as chemokine gene expression and neutrophilic alveolitis (59), and also TNF-α and IL-6 production (60).
Interestingly, two dipeptide boronic acid analogs that inhibit proteasome activity are currently undergoing clinical evaluation: PS-519 is being studied for the treatment of reperfusion injury following cerebral ischemia and myocardial infarction (61, 62). PS-341, associated with the inhibition of both IκBα degradation and NF-κB–dependent gene expression and concomitant anti-inflammatory effects in a rat model of polyarthritis and liver inflammation, recently entered clinical trials (63, 64). The therapeutic results of PS-519 and PS-341 and their role in inhibiting NF-κB should prove very interesting; whether targeting proteasome activity, involved in so many cellular functions, will provide a specific treatment for inflammation remains an open question.
Antisense Oligodeoxynucleotides
Antisense oligodeoxynucleotides constitute a potentially important family of therapeutic compounds for the treatment of a range of diseases (65). The use of antisense technology to inhibit expression of NF-κB proteins has been evaluated both in vitro and in vivo in a variety of experimental systems (Table 1⇑). Antisense targeting of the NF-κB p50 subunit can reduce IgM and IgG synthesis in B cells (66), and can significantly reduce maturation and proliferation of CD25/IL-2R T-cells (67). Antisense strategies to reduce expression of the p65 subunit greatly inhibits the expression of cell adhesion molecules in endothelial and smooth muscle cells in vitro (68). In vivo, antisense inhibition of p65 blocks tumor growth in nude mice, prolongs allo- and xenograft survival (69, 70), and alleviates septic shock in LPS-treated animals (71, 72). Clearly, the therapeutic potential of antisense molecules directed against NF-κB and the components required for its expression and activation warrants further investigation.
Oligodeoxynucleotides as Therapeutic Decoys
The ability of transcription factors to specifically recognize short nucleotide sequences even in the absence of surrounding genomic DNA has made it possible to design specific oligodeoxynucleotides as tools for manipulating gene expression in living cells. This strategy involves the intracellular delivery of cognate oligodeoxynucleotides, known as “transcription factor decoy” oligodeoxynucleotides, that bind to transcription factors and thereby preclude promoter activation. In experimental models of inflammation (Table 1⇑), NF-κB–specific oligodeoxynucleotides have thus been effective in attenuating the expression of IL-1α, IL-1β, IL-6, intracellular adhesion molecule 1, and vascular cell adhesion molecule 1 at both the transcriptional and translational level (73–78). The injection of NF-κB–specific oligodeoxynucleotides into hind ankle joints in a rat model of rheumatoid arthritis ameliorates the severity of paw swelling and joint deterioration (75); specifically, this treatment suppresses the synovial production of IL-1 and TNF-α. Similarly, in rat models of ischemia reperfusion, oligodeoxynucleotide decoys inhibited NF-κB and thereby precluded both neutrophil adherence and tissue IL-8 production (74, 79, 80). The inhibition of NF-κB by decoy oligodeoxynucleotides has also been effective in blocking cytokine and adhesion molecule expression as well as renal inflammatory responses in a mouse model of nephritis induced by TNF-α (81). Finally, local administration of NF-κB decoy oligodeoxynucleotides inhibits edema, cell migration, and COX-2 and inducible nitric oxide synthase expression in models of acute and immune-mediated inflammation in rats (82, 83).
Natural Compounds
For centuries, dietary and medicinal phytochemicals have been used as anti-inflammatory remedies, and considerable attention has focused recently on identifying the active components of these traditional preparations. Several compounds have been purified, and some have been shown to inhibit NF-κB at concentrations comparable to those of classical anti-inflammatory drugs (Table 1⇑) (84–102). Green tea polyphenols and resveratrol, a polyphenol present in red wine, inhibit NF-κB activation in vitro by blocking the activity of IKK (12, 84, 89). Green tea polyphenols also inhibit LPS-induced NF-κB activation and septic shock in mice (85); NF-κB activity in peripheral blood mononuclear cells during postprandial lipemia is reduced in humans after drinking red wine (88). Anti-inflammatory sesquiterpene lactones derived from various classes of medicinal plants also act, via inhibition of IκB phosphorylation, to block NF-κB activation in cell types ranging from T-cells and macrophages to fibrosarcoma and epithelial cells (91–96). Curcumin (i.e., a yellow pigment from turmeric) and capsaicin (i.e., a pungent component of red pepper that exhibits profound anticarcinogenic and antimutagenic activities) are potent inhibitors of IKK activity in several cell types (97–101). The application of curcumin onto the dorsal skin of mice significantly attenuates phorbol ester–induced NF-κB activation (102).
Cell-Penetrating Peptides
A novel approach to the development of selective and safe anti-inflammatory drugs comes from the prospect of using recombinant proteins or peptides to target pivotal inflammatory mediators or signal transduction pathways. This approach rests on a recent wealth of studies that have identified and characterized several effective peptide-based methods that allow membrane translocation of otherwise cell-impermeant peptide cargoes (103). Our expanding knowledge of the modular organization and structure of proteins, together with the biochemical identification and characterization of localized protein–protein interaction motifs, has prompted the design and exploitation of small peptide molecules that can interfere with protein–protein interactions (104, 105).
An early attempt to develop cell-penetrating peptides to block NF-κB activity used a forty-one–residue peptide consisting of the nuclear localization sequence of NF-κB (p50 subunit) fused with the signal sequence of Kaposi's fibroblast growth factor (106). The resulting peptide (named SN50; see Table 1⇑) effectively inhibits LPS- and TNF-α–induced nuclear translocation of NF-κB in several different cell lines (106–110), and also mitigates inflammatory responses in vivo (82, 83). However, subsequent analysis has demonstrated that SN50 is neither specific for p50 nor NF-κB in general (108, 111); SN50 also blocks the nuclear localization of the transcription factors STAT, AP-1 and NFAT in primary T-cells by competing for proteins generally involved in nuclear import (111).
In another attempt to target the nuclear localization of NF-κB, a peptide inhibitor was developed consisting of two nuclear localization sequences positioned on either side of the fibroblast growth factor translocation peptide (112); the peptide inhibitor was synthesized using D-amino acids to minimize susceptibility to proteases (denoted simply as “NLS” in Table 1⇑). Like SN50, this peptide proved effective for the inhibition of NF-κB-dependent gene expression in vitro and ameliorated responses in mouse models of septic shock and inflammatory bowel disease. However, the effect of this peptide on other transcription factors remains to be fully evaluated.
Studies in our laboratory investigating the structure and function of the IKK complex identified the region present in both IKKα and IKKβ that facilitates their interaction with NEMO. This NEMO-binding domain (NBD) consists of a hexapeptide sequence within the extreme C termini of both IKKα and IKKβ. In order to block the assembly of the IKK complex so as to preclude NF-κB activation, we made a cell-penetrating peptide by fusing the NBD sequence of IKKβ with penetratin, a membrane translocation sequence derived from the Drosophila antennapedia protein (103). The resulting peptide not only blocks the association of NEMO with IKKβ and with IKKα (F. D'Acquisto, M.J. May, and S. Ghosh, unpublished observations), but also inhibits TNF-α–induced NF-κB activation in HeLa cells (113). Further studies, in which the peptide inhibited E-selectin expression in HUVEC and NO production in macrophages (113), together with several recent reports from other workers (114–117), demonstrate the potential of the peptide to function as a potent anti-inflammatory agent. This possibility has been tested experimentally in various animal models of inflammation, including phorbol ester–induced ear edema and zymosan-induced peritononitis (113), as well as LPS-induced septic shock, and carrageenan-induced paw swelling (F. D'Acquisto, M.J. May, and S. Ghosh, unpublished data); in each case, the NBD–penetratin peptide ameliorates the inflammatory response. Drugs targeting the IKK-NEMO interaction may therefore be of clinical importance for the control of inflammation, and as the NBD is only six amino acids long, it should be possible to design effective peptidomimetic compounds that disrupt the IKK complex assembly.
Although NF-κB represents an extremely attractive target for therapeutic intervention, one critical question is how to achieve anti-inflammatory selectivity by targeting a transcription factor that functions in so many biological systems. In particular, the role of NF-κB in the induction of several anti-apoptotic factors suggests that its complete inhibition would result in unforeseen and harmful side effects. However, the very complexity of the pathways involved in NF-κB signaling might offer the opportunity to develop specific inhibitors, with distinct mechanisms of action, that would not globally inhibit NF-κB. For example, recent studies demonstrate that NF-κB plays a key role in critical, evolutionarily conserved signaling pathways that prime the adaptive immune response (118). In the pathway that is initiated by ligand binding to Toll-like receptor 4 (TLR4), for example, a recently identified adapter protein named TIRAP (Toll-interleukin 1 receptor domain–containing adapter protein) was found to contain a specific sequence by which it associates with TLR4 (119). A peptide consisting of this interaction sequence fused with the penetratin peptide effectively blocks the association of TLR4 with TIRAP and thereby inhibits LPS-induced NF-κB activation. In contrast, the TIRAP peptide inhibits neither CpG-nor IL-1–induced modes of NF-κB activation (i.e., modes that are not dependent on TIRAP) (119). Taken together these data suggest that the TIRAP peptide may be a specific inhibitor of LPS-induced NF-κB–activation pathways.
LIMITATIONS AND FUTURE CHALLENGES
After nearly fifteen years of intense study, it is now clear that NF-κB represents a realistic molecular target for the development of effective anti-inflammatory therapies (Figure 3⇓). The discovery that various NSAIDs and GCs are potent inhibitors of NF-κB has dramatically influenced our understanding of the molecular mechanisms underlying the anti-inflammatory actions of these drugs. This new understanding, however, does not provide a meaningful strategy by which the beneficial pharmacological effects of these agents can be separated from their undesirable side effects. For example, whereas inhibition of COX by NSAIDs decreases the production of all PGs and thromboxane, thereby alleviating inflammation, the price paid for these anti-inflammatory, antipyretic, analgesic, and cardiovascular effects is the danger of gastrointestinal side effects. Thus, a number of challenges must be addressed before considering inhibitors of NF-κB as anti-inflammatory drugs.
A variety of drugs that inhibit NF-κB have been classified according to their molecular targets. Several intermediate steps (denoted at right) in proinflammatory signal transduction pathways can be targeted by inhibitors of NF-κB (indicated on left). The dimeric nature of NF-κB, consisting of 50-kDa and 65-kDa subunits, is indicated by two circles. Synthetic, cell-penetrating peptides, such as those derived from TIRAP (Toll-interleukin 1 receptor domain-containing adapter protein), the NEMO-binding domain (NMD) of the IKKβ protein, the nuclear localization signal of the p50 subunit of NF-κB (SN50), and other nuclear localization signals (NLSs), are discussed in the text. (Other abbreviations: ROS, reactive oxygen species; NEMO, NF-κB essential modulator; IKK, IκB kinase; IκB, inhibitor of NF-κB; TFD-ODNs, transcription factor decoy oligodeoxynucleotides.)
The multiplicity of transcription factors that regulate any single gene, and the wide range of target genes that are controlled by NF-κB, present significant limitations to the use of oligodeoxynucleotides in antisense and transcription factor decoy strategies. Specificity becomes an even greater challenge when target gene expression is to be inhibited in only a single organ or tissue type. In addition, all of the experimental hurdles, such as reagent lability, that characterize the use of oligonucleotides as therapeutics will continue to plague such attempts to target NF-κB.
Peptide therapy seems to provide a promising new outlook for the development of anti-inflammatory drugs. Previous studies using soluble receptors or neutralizing antibodies have demonstrated impressive efficacy in clinical trials for chronic inflammatory diseases. Thus, anti-TNF-α antibodies and soluble TNF receptor preparations show striking anti-inflammatory potential in clinical studies of rheumatoid arthritis (see Song et al., in this issue)(120). However, the problems of protein delivery, immunogenicity, and cost of treatment have limited the realistic prospect of whole proteins for therapy. In this regard, the potential use of peptide therapy would be a tremendous step forward, because small molecules are much easier to synthesize, present a very low immunogenic profile, and are relatively inexpensive. Furthermore, small peptides have the potential to selectively block specific signaling pathways that are dependent on NF-κB activation without affecting all NF-κB–dependent functions. As is the case for proteins, a significant challenge in developing therapeutic peptides and peptidomimetic compounds is their effective delivery to the patient. Fortunately, the number of membrane-penetrating peptides that can be used to carry biologically active peptide cargo rapidly and efficiently into cells is expanding.
Acknowledgments
Research in the authors' laboratory is supported by grants from the National Institutes of Health, the American Heart Association, and the Howard Hughes Medical Institute.
- © American Society for Pharmacology and Experimental Theraputics 2002
References
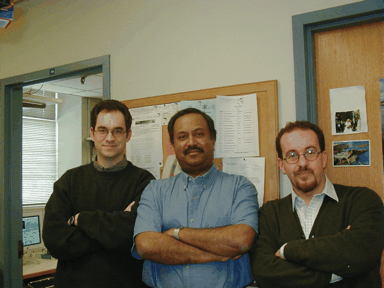
Fulvio D'Acquisto, PhD, (right) and Michael J. May, PhD, (left) are Research Fellows in the laboratory of Sankar Gosh, PhD, who is Professor of Immunobiology and Molecular Biophysics and Biochemistry at Yale University, and Associate Investigator of the Howard Hughes Medical Institute.