Signaling Actions of Electrophiles: Anti-inflammatory Therapeutic Candidates
Abstract
Over the past several years, research on biologically relevant electrophiles has been replete with new insights, expanding our understanding of the roles electrophiles play in vivo. Importantly, many electrophiles can form reversible covalent adducts with both proteins and small-molecule thiols in cells. This post-translational protein modification has important ramifications, including changes in protein enzymatic activity, the transduction of signals within and between cells, and alterations in gene expression. Electrophiles modulate a variety of cellular signaling processes that are involved in several major diseases with inflammatory components. The electrophilic fatty-acid derivatives discussed in this work are naturally occurring products of redox reactions and enzymatic activity. Furthermore, several of these electrophilic species and their derivatives represent potential therapeutic candidates.
Introduction
During inflammation the oxidative environment and the enzymatic production of lipophilic signaling mediators (e.g., prostanoids and nitric oxide; •NO) both contribute to the generation of reactive electrophilic species. These species are emerging as key mediators of inflammatory processes and new electrophiles are still being discovered. Electrophiles, such as nitroalkene derivatives of fatty acids (NO2-FAs), are formed independently of known enzymatic pathways and can be products of pH-dependent, NO2−-mediated nitration. Regardless of their path of origin, many electrophiles generated during inflammation can be considered “soft” electrophiles (1) that can react reversibly with nucleophilic biomolecules by Michael addition (i.e., the addition of a nucleophile to either an alkene or alkyne that is conjugated to an electron-withdrawing functional group). This reversible reactivity confers electrophiles with signaling capabilities that inform the cell of its redox status or external environment.
Moderate exposure to oxidative and electrophile-instigated stress stimulates a cell to orchestrate the expression of cell survival proteins and thus better prepare for future insult. Conversely, acute, extreme oxidative and electrophile-induced stress trigger cell damage, often ending in senescence, apoptosis, or necrosis. Cellular protective mechanisms for oxidative and electrophile-induced stress include the activation of transcription factors, the repair of DNA damage, and increased expression of protective proteins such as antioxidant enzymes, glutathione (GSH), and heat shock proteins. Genes encoding many of these defensive products contain an electrophile response element/antioxidant response element (EpRE/ARE). The transcription factor Nrf2 regulates the expression of EpRE/ARE-dependent genes and Nrf2 activity is determined by the state of its redox-sensitive inhibitor, Keap1 (kelch-like ECH-associated protein 1). Herein, the origins and roles of fatty acid-derived and other lipophilic electrophiles in inflammation and disease are explored.
Characteristics of Electrophiles
The broad definition of an electrophile is a molecule having one or more electron-poor atoms, which can accept electrons from electron-rich donor molecules (nucleophiles). Thus, cations (e.g., Hg2+, Cd2+, Zn2+), polarized neutral molecules, polarizable neutral molecules (e.g. Cl2 and Br2), oxidizing agents, and some Lewis acids would all be included in this definition. Biologically relevant electrophiles are generated during tightly controlled metabolic processes or during dysregulated pathological processes as byproducts of oxidation. Following generation, electrophiles may contribute to pathogenesis by altering cellular functions directly via the modulation of signaling pathways, or indirectly, by covalently modifying cellular macromolecules, depleting the cell of reductants (2). Thus, when produced in excess, electrophiles are associated with the development and progression of a number of diseases including neurodegeneration (1), cancer (3–5), atherosclerosis (6), Alzheimer’s disease (7), and chronic inflammation (8). Alternatively, in lower concentrations in the cell, electrophiles can induce protective effects against further insult by activating the expression of genes involved in xenobiotic detoxification and the antioxidant response.
Electrophiles can serve as signaling mediators in organisms spanning the Eukaryota domain. Carbon atoms are often rendered electrophilic by conjugation to electron-withdrawing functional groups. Often, specific functional groups determine the reactivity of the electrophilic moiety (9). Two prominent examples of these electron withdrawing groups are α,β-unsaturated carbonyls and nitroalkenes, in which the β-carbon (if it is bound to at least one hydrogen atom) is electrophilic and hence susceptible to nucleophilic attack (Figure 1). Although electrophiles can be derived from many sources, this review focuses on fatty acid–derived electrophilic products of oxidation and/or nitration reactions.
The β-carbons of nitroalkenes and α,β-unsaturated carbonyls are electrophilic. The chemical structures of a nitroalkene (left) and an α,β-unsaturated carbonyl (right) are represented above, in which “*” indicates an electrophilic β-carbon.
Electrophiles modulate cell survival at several levels by chemically reacting with nucleophilic nucleic acids, amino acid residues (cysteine, lysine, and histidine) of proteins, and other small molecules (10–12). For example, electrophiles not only directly modulate protein function by reacting with thiol (R-SH) groups on cysteine (13, 14), but can also diminish the pools of small-molecule thiols and cellular reductants such as GSH (15, 16), thus indirectly interfering with basic homeostatic functions. Both electrophiles and reactive oxygen species (ROS) alter the cellular redox state and share other comparable features; both can be produced by either non-enzymatic or enzymatic processes (depending on the species in question). In both processes, the amounts of ROS and electrophiles produced are metabolically controlled in healthy cells; low concentrations of these species induce the expression of cell survival genes, and, in some cases, prime the cell to survive periods of stress (13, 17). In contrast, under pathological conditions, electrophiles and ROS are often produced in excess and accelerate cell damage by chemically modifying nucleophilic atoms in cellular molecules through uncatalyzed reactions (9, 18). Several recently emerging classes of lipid mediators can be formed by enzymatic or non-enzymatic oxidative processes and have demonstrated robust anti-inflammatory signaling activities. Among these are NO2-FAs, and α,β-unsaturated keto fatty acids [(e.g., oxo-eicosatetraenoates (oxoETEs), and neuroprostanes] (Figure 2).
NO2-FA
In addition to concentrating O2 and lipophilic nitrogen oxides (i.e., •NO and N2O3), the hydrophobic environment of biological membranes facilitates and accelerates nitration, oxidation, and nitrosation reactions. This hydrophobic partitioning influences the reaction rates and product profiles of •NO-derived species not only by promoting their accumulation, but also by providing an aprotic (i.e., incapable of being a proton donor) interior and unfavorable conditions for their hydrolysis (19). •NO can react with peroxidizing lipid mixtures to terminate radical chain propagation reactions and to yield nitrogen-containing lipid species (Figure 3) (20). Additionally, in the presence of •NO and nitrite, lipoxygenase-catalyzed oxidation of low-density lipoprotein (LDL) yields nitrogen-containing derivatives of oxidized lipids (21, 22). Thus, it is not surprising that nitrated lipids have now been identified in vivo.
Multiple mechanisms can lead to the nitration of lipids. A. Biologically relevant NO2-FA products resulting from reaction of PUFA with NOX and reactive oxygen species. B. Reactive intermediates from autoxidation of •NO and acidification of nitrite. Under aerobic conditions •NO can react rapidly with O2 to form •NO2. When the O2 concentration is significantly lower than atmospheric conditions, •NO2 and •NO can be in equilibrium with N2O3. In aqueous milieu, N2O3 can be in equilibrium with HNO2. Finally, HNO2 is in equilibrium with is conjugate base NO2− (pKa=3.35). As all of these reactions are reversible, their order and equilibria depend on the source and site of production of NOX, the concentrations of various intermediates, and their reaction with other biomolecules.
Free and esterified polyunsaturated fatty acids (PUFAs), such as linoleate, are especially predisposed to the formation of both reactive intermediates and bioactive products. Nitrated derivatives of oleic (OA-NO2), linoleic (LNO2), linolenic, arachidonic (AA-NO2), and eicosapentaenoic acids have been identified in mammals. Moreover, nitrated derivatives of unsaturated fatty acids are present in healthy human blood (23, 24), and nitrohydroxy derivatives of linoleate have been detected in healthy human plasma and lipoproteins (23, 25). The chemical properties of nitroalkenes contribute to their ability to release •NO and act as unique ligands for nuclear receptors. For example, •NO can be released from nitroalkenes by a modified Nef reaction—the decomposition of a nitroalkene to the resultant carbonyl species and a nitroxyl species (HNO)—or by the isomerization of nitrite derivatives that undergo homolytic scission and/or transition metal-catalyzed reduction (26, 27). Nitroalkene-protein adducts have been identified in healthy human erythrocytes and nitroalkenes inhibit the enzymatic activity of glyceraldehyde-3-phosphate dehydrogenase (GAPDH) by covalently adducting to Cys-149 (14). In terms of other nitroalkene-protein adducts, NO2-FAs: 1) inhibit tumor necrosis factor–α (TNFα)- and lipopolysaccharide (LPS)-induced macrophage activation by nitroalkylation of the 65-kDa subunit of nuclear factor–kappa B (NF-κB) (p65) (28); 2) inactivate xanthine oxidoreductase (29); and 3) activate Keap1-Nrf2-dependent gene expression (30).
α,β-Unsaturated Oxo-Derivatives of Fatty Acids
Electrophilic derivatives can be generated by non-enzymatic, free radical-mediated reactions or by enzyme-catalyzed reactions. Non-enzymatic generation of electrophiles occurs under oxidizing conditions in which ROS or lipid alkoxyl/peroxyl radicals initiate and propagate the oxidation of PUFAs. Termination of these reactions yields hydroxy- and hydroperoxy-lipid derivatives as well as fragments of peroxidized lipids, which can include short chain conjugated carbonyl products. In contrast, enzyme-catalyzed electrophile generation represents products of more controlled conditions that can be generated both during periods stemming from stress or normal metabolism. Under these circumstances, the production of many electrophiles begins with oxygenases. For example, fatty acids [e.g., arachidonic acid (AA)] and other lipophilic molecules are converted to hydroxyl species by cyclooxygenases (COXs) and lipoxygenases (LOXs) and can be further metabolized to electrophiles by dehydrogenases (31, 32). Two of the well-characterized electrophilic fatty-acid derivatives formed by these pathways are the α,β unsaturated keto electrophiles 15d-PGJ2 and oxoETEs.
Non-Enzymatic Generation of Reactive Electrophilic Species
Many electrophiles are also generated independently of enzymatic catalysis. Malondialdehyde (MDA) is one of the major non-enzymatic products of lipid oxidation reactions in both mammalian and plant systems. Because of tautomerization, MDA exists primarily in the enol form as an α,β-unsaturated aldehyde. In healthy plant leaves MDA is produced continuously (mostly from trienoic fatty acids), and although its concentration is relatively high, it is maintained despite rapid turn-over or sequestration by the cell (Figure 4) (9, 33). In humans, MDA is the product of lipid peroxidation and can be generated under pathological oxidative conditions, such as in macrophage-derived foam cells of atherosclerotic lesions (34). Other products of lipid peroxidation, 4-oxo-2-nonenal (4-ONE) and 4-hydroxy-2-nonenal (4-HNE), are electrophilic alkenals closely related to MDA (9) and formed under similar oxidizing conditions (35). Acrolein (2-propenal) can be generated from a variety of organic molecules (e.g., carbohydrates, lipids, amino acids, and biodiesel) during oxidation or combustion (36). Thus, acrolein is present in cooked foods, air pollution, and especially cigarette smoke (36). Not all electrophilic products of lipid oxidation are acyl chain fragments; an intact parent lipid may also be oxidized to an electrophile. For example, cyclopentenone neuroprostanes (i.e., A4/J4-NPs) are α,β-unsaturated keto products of docosahexaenoic acid (DHA) oxidation (Figure 5). A4/J4-NPs are formed by non-enzymatic lipid peroxidation in which an endoperoxide intermediate of DHA undergoes a series of reduction, rearrangement, and dehydration reactions to produce a cyclopentane ring with various functional groups (37–39).
Chemical structures of reactive electrophilic products of oxidation and electrophiles from dietary sources.
Xenobiotics and Dietary Sources of Electrophiles
Drugs or other xenobiotics and their metabolites can be converted to electrophiles. For example, raloxifene, a selective estrogen-receptor modulator (SERM), is converted to electrophilic quinoids, which can form adducts with nucleic acids, GSH, and proteins such as cytochrome p450 3A4 (CYP3A4) (40, 41). Additionally, non-steroidal anti-inflammatory drugs (NSAIDs) are metabolized, mainly by CYP3A4, to electrophilic quinoid intermediates, which covalently react with GSH and liver microsomal proteins (42).
Electrophiles can also be enzymatically formed in plants and subsequently consumed by animals. The increased production of electrophiles in plants can be in response to wounding, pathogenesis, and environmental stress. Although many electrophiles are considered secondary metabolites, some act as volatile hormones that ultimately alter gene expression in response to various stresses (9, 43). In higher plants, two common electrophiles, oxophytodienoic acid (the precursor of jasmonic acid) and (E)-2-hexenal, are produced by plant LOXs. Other phytochemicals with electrophilic capacity include members of the capsaicinoid, isothiocyanate, and triterpenoid families, as well as other secondary metabolites (Figure 6).
Capsaicinoids are a family of metabolites found in chili peppers that interact with mammalian capsaicin receptors [(transient receptor potential vanilloid-1 receptor (TRPV1)]. The metabolism of capsaicinoids by CYP enzymes produces electrophiles that can form covalent adducts with GSH (44). Capsaicinoid activation of TRPV1, a calcium channel expressed by sensory nerves, produces a burning sensation, hyperalgesia, erythemia, and irritation. Chronic exposure to capsaicinoids results in anti-inflammatory effects and long-term analgesia via desensitization of TRPV1 (45).
Isothiocyanates are another family of phytochemical electrophiles that are produced by Brassica vegetables (e.g., broccoli, cabbage, radish, etc.). Members of this electrophile family signal through the Keap1-Nrf2 pathway and through transient receptor potential-A1 (TRPA1) ion channels, which are involved in the perception of stimuli including cold temperatures and pungent compounds. Allylisothiocyanate is one of the major components responsible for the pungent flavor of mustard seeds and horse-radish (46). Broccoli also produces isothiocyanates such as sulforaphane (47). Finally, wasabi, although not of the same genus, has three isothiocyanates contributing to its flavor: 6-methylthiohexyl isothiocyanate, 7-methylthioheptyl isothiocyanate and 8-methylthiooctyl isothiocyanate (48). Cinnamaldehyde, found in the bark of cinnamon trees, contains an α,β-unsaturated aldehyde, enabling it to act as a Michael-addition acceptor and as a potent activator of TRPA1 (46).
A family of electrophilic triterpenoids containing a side chain with two α,β-unsaturated carbonyl groups, avicins, are produced by an Australian desert tree, Acacia victoriae. Avicins activate the antioxidant response pathway and may suppress carcinogenesis (49–51). Synthetic electrophilic triterpenoid derivatives of olea-nolic acid also display anti-inflammatory, antioxidant and anti-proliferative effects (52–55).
Electrophile Reaction with Biomolecules by Michael Addition and Schiff Base Formation
Michael-reaction acceptors are molecules containing charged or polarized functional groups conjugated with alkenes or alkynes. The functional groups most commonly associated with Michael-reaction acceptors in descending order of electron-withdrawing strength are: nitro groups (NO2), α,β-unsaturated carbonyl groups (i.e., aldehydes and ketones), and esters (56). The reactivity of a Michael acceptor with nucleophiles is positively correlated with the strength of the electron-withdrawing group(s) involved (Table 1 and Figure 1). In terms of signaling, a key property of Michael acceptors is that the majority can react reversibly with thiols, allowing for both signal initiation and termination (56).
Second-Order Rate Constants for Oxidants and Electrophiles Reaction with the Thiol of GSH.
The strength of an electrophile’s electron withdrawing group partially determines the rate of Michael addition reaction. As α,β-unsaturated oxo-derivatives of fatty acids, 15-deoxy-Δ-12,14-prostaglandin J2 (15d-PGJ2) and prostaglandin A2 (PGA2) both have a second-order rate constant ~0.7 M−1s−1 for reaction with GSH (57). In contrast, NO2-FAs react much more rapidly with GSH; the second-order rate constant for OA-NO2 reaction with GSH is ~183 M−1s−1 (57). These reaction rates are important to consider in terms of overall reactivity with nucleophilic biomolecules such as cysteine residues of proteins. It should be noted, however, that in the case of GSH, the reaction rates for both 15d-PGJ2 and PGA2 may not represent what is observed in vivo because glutathione-S transferases (GSTs) catalyze the adduction of GSH to electrophilic compounds. This can serve to equalize the ability of electrophiles with different reactivities to form adducts with GSH. The thiol group on the cysteine residue in GSH has a pKa of 9.2 (62), limiting spontaneous reaction with electrophiles or ROS at physiologic pH ranges. However, the high concentration of GSH in cells [~1–8 mM in mammalian tissue (63)] and GST-catalyzed reactions will significantly increase specific sites of reaction and of electrophilethiol adduction rates. Ultimately, the reaction rate of a particular electrophile will influence its ability to effect signaling or cell damage by the formation of adducts with cellular nucleophiles.
Another major factor that determines the rate of a Michael addition reaction is the pKa of the thiol involved. The Michael addition reaction is favored by deprotonation of the thiol to the more nucleophilic thiolate (R-S−) species. Thus, the pKa and the consequent abundance of the thiolate ion will determine a sulfhydryl’s rate of reaction with electrophiles. In the case of both free cysteines and those cysteines incorporated into proteins, their pKas are generally around 8.5 (58), indicating a low proportion of thiolates and, subsequently, mild reactivity at physiologic pH. The pKa of cysteine thiols can be significantly lowered, however, by proximity to basic amino-acid residues such as lysine or arginine (59). Protein structure can also affect the rate of a Michael addition reaction in terms of sterically hindering the electrophile from association or dissociation with nucleophiles.
Michael addition acceptors can also react with the imidizole nitrogen of histidine residues and the ɛ-amino nitrogen of lysine residues. Furthermore, carbonyl-containing electrophiles can react with lysine residues or free N-terminal amino groups to form Schiff base products. In some cases, bifunctional electrophiles (e.g., 4-ONE) form Michael adducts with one amino acid residue and induce protein cross-linking by forming a Schiff base with the amines of lysine residues (60, 61). By its nature, the Michael-addition reaction is reversible, whereas Schiff base products are more stable and much less prone to reversible reactivity.
Modification of Phospholipids and Nucleotides
Electrophiles react with nucleophilic biomolecules including phospholipids, nucleotides, and amino-acid residues in proteins. For example, rabbit lenses exposed to oxidative stress in vitro display time-dependent cross-linking between MDA and the amino groups of phospholipids (e.g., phosphatidylserine and phosphatidylethanolamine) (64). Electrophiles also react with DNA, forming adducts with deoxyguanosine, deoxyadenosine, deoxy-cytidine (4). Acrolein forms adducts with deoxyguanosine by a Michael-type addition, thus yielding 1,N2-propanodexoyguanosine in vitro (65). Additionally, acrolein and crotonaldehyde form 1,N2-propanodexoyguanosine diastereomers and effect base substitution, with G-to-T transversions being the most common. These adducts can cause, for example, a higher incidence of miscoding in human xeroderma pigmentosum A cells (66, 67) and have been observed in the DNA of liver tissues from healthy humans and rodents (68). Moreover, during increased oxidative stress, owing to the elevated expression of COX-2, ONE adducts on DNA (heptanone-etheno-2′-deoxyguanosine) were detected in rat intestinal epithelial cells (69). Finally, bis (2-chloroethyl) sulfide (also known as sulfur mustard or mustard gas), a lipophilic and particularly strong electrophile, forms covalent adducts with DNA (as well as RNA and protein), causing DNA damage and fragmentation. In mice and humans, nucleic acid alkylation by bis (2-chloroethyl) sulfide contributes to severe toxicity and necrosis of multiple tissues including the liver (70).
Modification of Proteins and Protein Cross-Linking
Electrophiles post-translationally modify proteins by adducting to cysteine, histidine, and lysine residues, thus modulating protein function, subcellular location, and turnover. A revealing example of all three of these actions is the modulation of Keap1-Nrf2 signaling by electrophiles. Electrophilic protein modifications can also interfere with cytoskeletal structure; in vitro actin polymerization can be disrupted by 15d-PGJ2 adduction to actin at Cys374 (71).
Electrophile-protein adduct formation can modulate the activity and trafficking of redox-sensitive proteins. OA-NO2 forms reversible adducts with glyceraldehyde-3-phosphate dehydrogenase (GAPDH) at the catalytic Cys149 (as well as other residues), thus inhibiting GAPDH activity and increasing its affinity for lipophilic environments (i.e., membranes) (14). Biotinylated-15d-PGJ2 forms adducts with thioredoxin-1 (Trx1) at Cys35 and Cys69 in vitro as determined by mass spectrometry (72). The enzyme responsible for maintaining a population of reduced Trx, thioredoxin reductase (TrxR), is crucial for the proper structure and function of several redox-sensitive proteins, including p53. TrxR is especially susceptible to adduction by electrophiles because of the low pKa (5.2) of a selenocysteine in its C-terminal sequence (73). This selenocysteine residue, although not present in the TrxR active site, is necessary for catalytic activity (74). In RKO and HCT 116 cell lines, adduction of PGA1 amidopentyl biotin (and other α,β-unsaturated carbonyl compounds such as HNE) to TrxR occurs concurrently with inhibition of p53 function (3).
Electrophiles that can undergo oxidation-reduction cycling (quinones) and bifunctional electrophiles (e.g., HNE and ONE) readily generate intermolecular or intramolecular protein cross-links. Both the functional group and microenvironment determine the pKa of nucleophilic amino-acid residues, with lower pKas promoting adduct formation via nucleophilic attack. Structural motifs in proteins, such as cytochrome c, also determine the susceptibility and types of adduct formation with amino-acid residues (75). For example, electrophiles can cross-link solvent-exposed lysine residues in close proximity to each other, such as in the case of benzoquinone, which can adduct with proximal lysine residues (Lys25-Lys27 and Lys86-Lys87) of cytochrome c (75).
Electrophile Signaling
The unique properties of electrophiles confer multiple signaling capabilities (Figure 7); however, each electrophile may effect distinct signaling depending on reactivity and structure. For example, sets of genes that are activated by one type of electrophile may partially or extensively overlap with some of those activated by another. As Farmer notes (9), “…Transcriptome responses induced by treatment with one particular electrophile do not necessarily indicate that this [electrophile] is the bona fide regulator of the genes in question and indicates only that the genes are sensitive to [electrophiles].” The vast majority of electrophiles can modulate transcription through the Keap1-Nrf2 pathway, which regulates the EpRE/ARE. Related to this issue, EpRE-independent pathways of electrophile signaling and post-translational protein modification are also addressed.
Modulation of cellular signaling mechanisms by electrophiles. Electophiles (E) can act at many loci within the cell. A. At the cellular membrane, electrophiles can modulate ion channel activity (e.g., adduction to essential cysteine residues of the TRP channel, thereby activating TRP signaling). Some adducts can be sequestered by reaction with glutathione (GSH), and GS-electrophile adducts can undergo further thiol exchange reactions or exit the cell via a multidrug resistant protein (MDR) mechanism. B. Cytosolic proteins can react with electrophiles, inducing changes in protein structure, activity, subcellular localization, or stability. An example of these outcomes is the modulation of protein kinase signaling by electrophiles and subsequent modulation of protein activity or gene regulation (see panel C). Electrophiles also affect signaling by influencing mitochondrial respiration and redox status, for example by reacting with cytochrome c. C. In the nucleus, transcription factors can react with electrophiles to affect the transcription of genes involved in cell survival, metabolism, and differentiation. During severe oxidative conditions, electrophiles can also form adducts with DNA and promote miscoding.
Signaling Pathways Not Directly Related to the Electrophile Response Element
Electrophiles modulate several cell signaling pathways, including the heat shock response, pro-apoptotic events, kinase/phosphatase activities, and nuclear transcription factor function. Heat shock proteins (Hsps) are one of the cellular targets of electrophiles. HNE, generated during oxidative stress, covalently modifies cysteine residues on Hsp72 (Cys267) and Hsp90 (Cys572), as confirmed by LC-MS/MS. In the case of Hsp72 (but not Hsp90), HNE adduction with Cys267 inhibited its ATPase activity (76, 77). HNE also disrupts the interaction between Hsp70–1 and heat shock factor 1 (HSF1), thus allowing HSF1 translocation to the nucleus and subsequent activation of transcription of heat shock response genes (78). In greater concentrations, electrophiles can also induce pro-apoptotic signaling. For example, HNE induces time- and concentration-dependent apoptosis via activation of the c-Jun N-terminal protein kinase (JNK) pathway. HNE activates JNK by first activating apoptosis signal-regulating kinase (ASK1), which phosphorylates stress-activated protein kinase (SEK1), an upstream kinase of JNK (79).
Protein kinase-regulated signal transduction, such as the extracellular-signal regulated kinase-1/2 (Erk-1/2) pathway and the p38 mitogen-activated protein kinase (p38 MAPK) pathway are modulated by electrophiles. HNE decreases Erk-1/2 phosphorylation and activity in primary rat hepatocytes by adducting to His178 on Erk-1/2 (80). Additionally, HNE potently induces COX-2 gene expression in RAW264.7 cells via activation of the p38 MAPK pathway and stabilization of COX-2 mRNA (6). Finally, protein serine–threonine phosphatase 2A (PP2A) shows decreased activity in HEK 293 cells upon alkylation of six cysteine residues by the biotin-labeled Michael addition acceptor, biotinamido-4-[4′-(maleimidomethyl) cyclohexanecarboxyamido]butane (81).
Electrophiles also modulate the activities of nuclear receptors and transcription factors which are involved in the regulation of inflammation such as NF-κB. Many electrophiles inhibit NF-κB signaling at one or more points in the pathway. For example, in RAW264.7 murine macrophages, A4-NP, a cyclopentenone neuroprostane, significantly inhibits LPS-induced iNOS expression and subsequent •NO production (as measured by nitrite levels in the culture media) via inhibition of NF-κB signaling (38). The expression of COX-2 was also reduced though not to the same extent as iNOS, which was attributed to the regulation of COX-2 by several pathways in addition to NF-κB. In luciferase reporter assays, A4-NP inhibited NF-κB-mediated transcription induced by LPS, as well as by TNFα and interleukin- (IL)-1β, all of which activate the inhibitor of κB kinase (IKK) and NF-κB via different receptors and mechanisms (38). Further probing into the NF-κB pathway identified adduction of A4-NP to Cys179 of IKK as the likely cause of inhibition; adduction to IKK prevents the phosphorylation and degradation of IκBα, which binds to the NF-κB p65 subunit and prevents NF-κB translocation to the nucleus (38). NO2-FAs also inhibit LPS-stimulated NF-κB-mediated transcription in RAW264.7 cells transiently transfected with a NF-κB-luciferase reporter construct. The use of biotinylated NO2-FAs, immunoprecipitation, and mass spectrometry-based strategies further identified p65 as the covalently modified protein and the most likely point of NO2-FA-mediated inhibition of the NF-κB pathway (28).
The synthetic 14-A4-NP confers anti-inflammatory properties to RAW264.7 macrophages that are attributed to its electrophilic reactivity with Cys179 of IKKβ; the mutation of Cys179 diminished A4-NP-mediated inhibition of NF-κB-dependent transcription (38). The inhibition of iNOS expression by A4-NP was dependent on its electrophilic moiety; the formation of GSH adducts or the reduction of the keto group with NaBH4 both significantly diminished the ability of A4-NP to inhibit LPS-induced •NO production (38). Despite its ability to activate PPARγ, the anti-inflammatory effects of A4-NP appear to be peroxisome proliferator-activated receptor–γ (PPARγ)-independent. It is important to note, however, that this conclusion was only based on the inability of PPARγ antagonists (GW9662 and T0070907) to reverse A4-NP-mediated inhibition of LPS-induced nitrite production (38).
Electrophile Response Element–Mediated Signaling
The EpRE is a cis-acting element found in the regulatory region of over 200 genes (12) that assist in the response to oxidative or xenobiotic stresses. Some of the xenobiotic/drug-metabolizing proteins and antioxidant proteins under EpRE regulation are GSTs, NAD(P)H-quinone oxidoreductases 1 (NQO1), hemeoxygenase-1 (HO-1), subunits of γ-glutamylcysteine synthetase (γ-GCS), glutamate-cysteine ligase (GCL), and thioredoxin (2, 82).
The metabolism of xenobiotics has been described as a two-phase process. During phase I, compounds are modified with new functional groups by oxidation, reduction and/or hydrolysis reactions. During phase II, the products of phase I are conjugated to molecules such as GSH or glucuronic acid, producing metabolites that are more hydrophilic and more easily exported and excreted (83). Inducers of the ARE/EpRE can be monofunctional or bifunctional. Bifunctional inducers increase the activities of both phase I and phase II enzymes, while monofunctional inducers only increase the activities of phase II enzymes. There are up to ten major chemical classes of monofunctional inducers and among these are Michael reaction acceptors (including electrophiles and divalent metal cations such as Hg2+, Cd2+, and Zn2+), hydroperoxides, phenolic antioxidants (BHA, BHT), 1,2-dithiole-3-thiones, and isothiocyanates. Monofunctional inducers typically activate phase II gene expression by reacting with sulfhydryl or disulfide groups, resulting in alkylation, oxidation, reduction, or thiol interchange. Thus, Michael reaction acceptors (electrophiles) are one subgroup of this inducer family (2, 56).
The Role of Nuclear Factor-Erythroid 2-Related Factor 2 (Nrf2) and Kelch-Like ECH Associated Protein 1 (Keap1) in Electrophile Response Signaling
One of the key transcriptional regulators of EpRE-dependent gene expression is the transcription factor Nrf2 [also termed chicken erythroid-derived CNC-homology factor (ECH)]. Nrf2 is turned over rapidly by the proteasome under basal conditions, but its half-life can be extended in the presence of electrophiles that induce the dissociation of Nrf2 and its partner Keap1. Typically, Keap1 recruits Cul3 (an E3 ubiquitin ligase) to the Keap1-Nrf2 complex, allowing the ubiquitination of Nrf2. Keap1 directly binds Nrf2 at an ETGE tetrapeptide motif in the evolutionarily conserved N-terminal domain (the Neh2 domain) of Nrf2, thus targeting Nrf2 for proteasomal degradation (84). By modifying Keap1, electrophiles and ROS prevent the ubiquitination of Nrf2 and thus stabilize newly produced Nrf2, which can then activate EpRE-dependent genes (85).
Some of the major and most-well-studied sulfhydryl sensors in cells are located on Keap1. Keap1 is a dimeric metalloprotein with five major domains and binds via its Kelch domain with the Neh2 domains of Nrf2 and actin (85). Murine Keap1 has twenty-five cysteines that are conserved in human Keap1, nine of which are especially nucleophilic owing to the presence of neighboring basic residues. Whereas twenty-three of these cysteine residues can react with electrophiles, only six (Cys151, Cys257, Cys273, Cys288, Cys297, and Cys613) are most often reported to react with electrophiles in cells (12, 56). Site-directed mutagenesis studies have demonstrated that Cys273 and Cys288 in the IVR of Keap1 (but not Cys257 or Cys613) are required for Keap1-mediated ubiquitination of Nrf2 and subsequent Nrf2 repression (2, 56, 86). Additionally, Cys151 in the Keap1 BTB domain is required for inhibition of Keap1-dependent degradation of Nrf2 by sulforaphane and oxidative stress (86).
Electrophiles as Therapeutic Candidates
Fatty acid-derived electrophiles, formed by oxidative, inflammatory, and detoxification events, can in turn mediate the progression or resolution of these events. Many diseases with an inflammatory component display significantly increased levels of electrophiles. For example, cyclopentenone neuroprostanes are found in frontal cortex brain tissue samples from Alzheimer’s disease patients at nearly three times the concentration normally observed in age-matched controls (38). Furthermore, increased amounts of oxoETEs are detected in the lungs of patients with severe pulmonary hypertension (87). Also the formation of NO2-FAs has been shown to be increased in mitochondria during ischemia reperfusion (88). Because all three of these electrophilic lipid derivatives display potent anti-inflammatory effects, it is viewed that to some extent that these species are also involved in the modulation of, if not protection from, the inflammatory events that form them.
For example, nitro-fatty acids induce anti-inflammatory gene expression, improve vascular function, attenuate pro-inflammatory PMN and macrophage functions, and, when administered in vivo, induce beneficial physiological responses such as protection from neointimal hyperplasia and cardiac ischemia-reperfusion injury (89–93). This and other data support that unsaturated fatty acid–derived electrophiles hold promise as beneficial therapeutic agents—a supposition reinforced by many of the actions of dietary omega-3 fatty acids (94). Once the effects of chronic lipid electrophile administration are more fully understood from pharmacokinetic and toxicological perspectives, new therapeutic strategies can be developed for modulating dietary and endogenous electrophile levels and applications of these compounds in the treatment of acute and chronic inflammatory diseases.
- Copyright © 2010
References

Bruce Freeman, Ph.D., received his doctorate in Biochemistry from University of California, Riverside, and performed postdoctoral studies and faculty service at the Duke University Medical School. He was then a Professor in the departments of Anesthesiology and Biochemistry and then served as the Director for the Center for Free Radical Biology at the University of Alabama, Birmingham. Since 2006, Bruce Freeman has been serving as the UPMC/Irwin Fridovich Professor and Chairman of the Department of Pharmacology and Chemical Biology at the University of Pittsburgh. His laboratory pioneered the concept that nitric oxide has cell signaling and pathogenic actions modulated by oxygen-derived reactive inflammatory mediators. His laboratory also discovered that nitric oxide-dependent reactions with unsaturated fatty acids yield nitrated lipid products that manifest potent receptor-dependent metabolic and anti-inflammatory signaling actions. Their discovery of nitric oxide reactions with products of eicosanoid synthesis, oxidases and peroxidases has also revealed clinically significant mechanisms of catalytic nitric oxide consumption that occur during inflammation. His research group’s most current observations of peroxynitrite, peroxidase and nitro-fatty acid-induced post-translational protein modification further underscore the significance of redox reactions in drug discovery and the regulation of cell and organ function. Dr. Freeman acknowledges financial interest in Complexa, Inc. E-mail freerad{at}pitt.edu; Fax: (412) 648-2229.
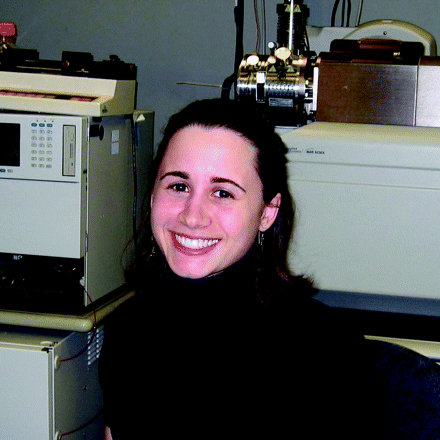
Alison Groeger, Ph.D., received her undergraduate training at Rhodes College. She performed her graduate training in Molecular Pharmacology, with Bruce Freeman at the University of Pittsburgh. Her dissertation focused on the biologically relevant formation and signaling properties of electrophilic omega-3 fatty acid derivatives. E-mail agroeger{at}pitt.edu